At the Canadian Geodetic Survey (CGS), we define, maintain, improve and facilitate access to the Canadian Spatial Reference System (CSRS), which establishes the reference for latitude, longitude, height and gravity in Canada. If you’re a surveyor, geoscientist, engineer or other professional, you might rely on the CSRS to provide a consistent approach to activities such as mapping, land surveying, engineering, water management and more.
How we define and access the CSRS has changed dramatically over time. That’s largely due to how technology has evolved, particularly the introduction of global navigation satellite systems (GNSSs) such as the global positioning system (GPS). We also know that the Earth is dynamic and continually changing. The CSRS has needed to evolve in order to provide a consistent reference for data collected at different epochs.
Canadian Spatial Reference System (CSRS)
The CSRS is a collection of standards, models, data products and infrastructure supporting geospatial positioning in Canada. It includes the geometric, height and gravity reference systems. You can learn about each of these and access related tools below.
On this page
- Geometric reference systems
- From the North American Datum of 1983 (NAD83(Original)) to the North American Datum of 1983 of the Canadian Spatial Reference System (NAD83(CSRS))
- North American Datum of 1983’s (NAD83) relationship with the International Terrestrial Reference Frame (ITRF) and the World Geodetic System of 1984 (WGS84)
- The role of geodetic control networks
- Coordinate transformations
- Canadian Velocity Grid (CVG)
- Height reference systems
- Gravity reference systems
Geometric reference systems
Geometric reference systems provide a way to define latitude, longitude and ellipsoidal height. In Canada, the official geometric reference system adopted in most jurisdictions is the NAD83(CSRS). When we publish coordinates for geodetic control points in a reference system, we define what we call a reference frame. As measuring techniques improve and the physical environment changes, we publish new coordinates to update that reference frame.
From the North American Datum of 1983 (NAD83(Original)) to the North American Datum of 1983 of the Canadian Spatial Reference System (NAD83(CSRS))
NAD83 has undergone several updates since we first introduced it in 1986. It has evolved from a traditional, ground-based, horizontal control network called NAD83(Original) to a space-based, dynamic 3D realization called NAD83(CSRS). However, many geospatial datasets still exist today in NAD83(Original).
NAD83(CSRS) represents a dynamic 3D realization of NAD83(Original). Canada and the U.S. collaborated to redefine NAD83 in relation to the ITRF through a seven-parameter transformation using VLBI stations common to both systems. We keep NAD83(CSRS) aligned to the North American plate using the NNR-NUVEL-1A estimate of plate motion.
North American Datum of 1983’s (NAD83) relationship with the International Terrestrial Reference Frame (ITRF) and the World Geodetic System of 1984 (WGS84)
Since 1990, the most accurate and stable reference frames available are the successive versions of the ITRF produced by the International Earth Rotation and Reference Systems Service (IERS). The ITRF is a global reference frame, so unlike NAD83, it’s not fixed to any specific tectonic plate. The dynamic nature of this frame means that positions in ITRF change with time, and the coordinates refer to a date we call an epoch.
WGS84 is another global reference system we use in Canada. It was originally developed by the U.S. National Imagery and Mapping Agency for their GPS positioning system. Like ITRF, it is periodically updated with new versions, which are denoted by the GPS week number of the date it was put into use. The latest version was implemented in 2024 and is called WGS84(G2296). It’s aligned to ITRF2020 at the centimetre level.
The role of geodetic control networks
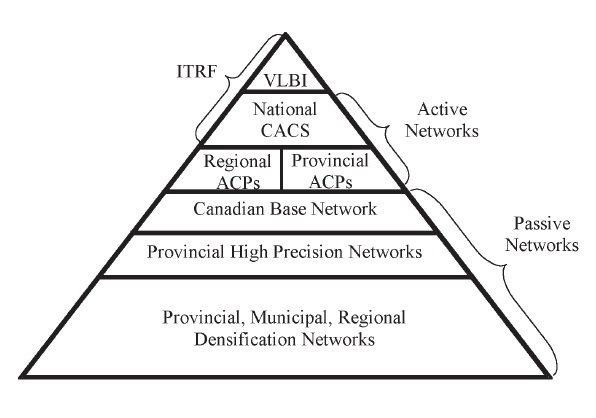
Figure 1: NAD83(CSRS) geometric reference frame
Text Version
This pyramid-shaped figure shows the hierarchy of geodetic control networks in NAD83(CSRS). At the bottom are the passive networks. These include provincial, municipal and regional densification networks as the foundation; then provincial high-precision networks; then the Canadian Base Network. On top of these are stacked the active networks, with regional and provincial active control points (ACPs) then the CACS. All of these are capped by the VLBI, which, along with the CACS, contributes to the ITRF.
Much like reference systems, geodetic control networks have evolved over the decades. Geodetic networks traditionally consisted of control monuments distributed across the Canadian landmass. The published coordinates for these monuments were the positioning standard, and surveyors used them to control their surveys. We refer to these networks of monuments as passive control networks.
GNSSs completely changed the way we realize, maintain and use reference frames. In the 1990s, CGS established the Canadian Active Control System (CACS), a sparse network of control stations which continuously track GNSS satellites. Along with data from international partners, it enables us to compute precise GNSS orbit and clock products. We use these products in the Canadian Spatial Reference System Precise Point Positioning (CSRS-PPP) to provide an accurate and efficient way to control surveys. We call these networks active control networks.
GNSSs and the extension of the geodetic reference frame into space also meant we had to more accurately monitor the Earth’s orientation in space. We did so by observing radio signals from quasars with VLBI. These activities marked our entry into the space-age of precise geodetic positioning. Figure 1 shows the hierarchy of geodetic control networks in NAD83(CSRS).
Very long baseline interferometry (VLBI)
VLBI is a technique used to determine very precise distances between radio telescopes in order to study the Earth and its precise orientation in space. It uses radio signals from quasars, which are at such great distances from the Earth that they provide fixed points in the sky that can be referenced to measure the Earth’s orientation, rotation and the motion of the rotation pole. VLBI’s precise measurements make it possible to detect even the slightest changes in the orientation of the Earth in space. VLBI also provides accurate measurements of very long distances that are critical to tying the global reference frame together. It is one of the space-based techniques — along with GNSS, Satellite Laser Ranging (SLR), and the Doppler Orbitography and Radiopositioning Integrated by Satellite (DORIS) system — that contribute to the realizations of the ITRF.
Active control systems
Active control networks, including the CACS, consist of permanently tracking GNSS stations — referred to as active control points (ACPs) — that record the signals received from GNSS satellites. These ACPs are equipped with a geodetic-quality GNSS antenna and receiver, and in some cases, an atomic frequency standard. CACS includes about 20 sites, some with multiple stations for monitoring their stability, that are used to tie NAD83(CSRS) to the ITRF. Several other public active networks exist in Canada, including regional networks operated by Natural Resources Canada (NRCan) for geoscience purposes, as well as provincial and municipal networks. We also use these public active networks to determine the motion of the Canadian landmass and estimate the velocity model described below.
Commercial real-time kinematic (RTK) networks
Although they’re not considered part of the CSRS, commercial RTK networks also operate in Canada. They consist of continuously tracking GNSS receivers used for either real-time network (RTN) or single-base (RTK) solutions. To preserve a nationally consistent geodetic infrastructure, we have entered into compliance agreements with interested commercial RTK network operators to integrate these RTK stations into the NAD83(CSRS) reference system in a consistent manner.
Take me to commercial RTK networks
Passive control networks
In Canada, passive control networks include the Canadian Base Network (CBN), the High Precision 3D (HP3D) Geodetic Network and the horizontal and vertical control networks.
The CBN links the existing land-based control networks to the CACS and helps us monitor the motion of the Canadian landmass. It’s a network of highly stable pillar monuments with forced-centering plates that are positioned three-dimensionally with GNSS to centimetre-level accuracy with respect to the CACS. The network consists of an array of pillars at an average spacing of 200 km in the built-up areas of southern Canada, 500 km in the middle regions of Canada and 1,000 km in the northern areas. We observe the CBN network stations periodically to help determine a crustal motion model, provided through the CVG.
The HP3D network consists of approximately 3,000 more traditional survey monuments and benchmarks that are observed with high-accuracy GNSS and tied to NAD83(CSRS).
We continue to publish coordinates for some 25,000 horizontal control points in NAD83(Original), and close to 81,000 vertical benchmarks in CGVD28 and CGVD2013 (see the section below on the vertical reference system for more information).
Notes about the horizontal and vertical control networks
- We developed the horizontal and vertical control networks independently from one another. So, for example, a survey monument that provided accurate horizontal coordinates was not normally connected to the vertical network, nor were vertical points related to the horizontal reference network.
- Although we continue to publish coordinates for these networks, they are no longer physically maintained and we haven’t observed them for decades. Therefore their published coordinates may not reflect their current positions.
Take me to passive control networks
Coordinate transformations
In collaboration with the U.S. National Geodetic Survey, we provide the official transformation parameters between NAD83(CSRS) and the various realizations of ITRF. You can download these from our coordinate transformations page. They’re also used in our coordinate transformation tool, TRX. We provide the transformation parameters (three translations, three rotations, one scale factor) for a reference epoch along with their rates of change with time.
You can also transform older geospatial datasets into NAD83(CSRS). Our National Transformation Version 2 (NTv2) tool allows for the horizontal transformation between the North American Datum of 1927 (NAD27), the Average Terrestrial System of 1977 (ATS77), NAD83(Original), and NAD83(CSRS) reference frames using binary grid shift files (in NTv2 format), where available.
Though there is a national grid shift file available to transform between NAD27 and NAD83(Original), many provinces have also produced grids to transform between various legacy systems and NAD83(CSRS) in their jurisdictions. We make these available on our coordinate transformations page. These grid shift files are built from the differences in coordinates at common points across the coverage area. The accuracy of the transformation depends on the distortion between the reference frames and the spatial distribution of the common points used to form the grid. It should be noted that, although our NTv2 tool allows for the transformation of legacy datasets to a more accurate reference frame, it does not improve the accuracy of these datasets.
Canadian Velocity Grid (CVG)
Although NAD83(CSRS) is fixed to the North American tectonic plate using a plate rotation model, there are regional and local 3D motions within the plate that we often need to account for. We’ve estimated a velocity grid that you can use to predict these motions throughout Canada. The latest version of the Canadian Velocity Grid is called NAD83v80VG. The grid is implemented in our tools for propagating coordinates between epochs (CSRS-PPP and TRX) and is available for scientific purposes, such as studies of sea-level change and the impact on coastal areas.
Height reference systems
Height reference systems such as the CGVD2013 and the CGVD28 define heights with respect to mean sea level (MSL). In 2015, we adopted CGVD2013 as our official height reference system, replacing CGVD28. We know it takes some time for stakeholders to switch over to the new system, so we continue to publish heights in the old system to assure a smooth transition. Although these systems both define heights with respect to MSL, they contain significant differences. Learn about each of these height systems below.
Canadian Geodetic Vertical Datum of 2013 (CGVD2013)
CGVD2013 is defined by the equipotential surface W0 = 62,636,856.0 m2/s2, which represents (by convention) the coastal MSL for North America. (This definition comes from an agreement between Canada and the U.S.) This new vertical datum is currently realized by the Canadian Gravimetric Geoid model of 2013 — Version A (CGG2013a). It provides the separation between the GRS80 ellipsoid and the equipotential surface described above in NAD83(CSRS), making it compatible with GNSSs such as GPS. The CGG2013a geoid model is referenced to epoch 2011.0 as this represents approximately the middle of the Gravity Field and Steady-State Ocean Circulation Explorer (GOCE) measurements used in the model. However, at this time we consider the geoid model static, i.e., the geoid heights do not change in time.
Geoid model
The geoid model allows you to convert GNSS ellipsoidal heights (h) to orthometric heights (H), i.e., heights above MSL. Orthometric heights are compatible with published elevations on benchmarks and topographical maps. They allow the proper management of water resources, as they include corrections for the Earth’s gravity field. The challenge lies in calculating the separation between the ellipsoid and geoid using gravity measurements. The separation between these two surfaces is called the geoid undulation or geoid height (N). CGS develops national geoid models, allowing users to interpolate the geoid height at a given position (φ, λ). An orthometric height can be calculated by subtracting the geoid height from the ellipsoidal height: H = h – N. Use our desktop or online GPS·H application to do these calculations.
Take me to the online GPS-H tool
Download the desktop GPS-H tool
The geoid, by definition, is the equipotential (level) surface that best represents, in a least squares sense, the global MSL. However, the underlying geoid for CGVD2013 is the equipotential surface (W0 = 62,636,856 m2/s2), which represents (by convention) the coastal MSL for North America. Like land, the ocean’s surface has a topography, although it’s much smaller at about +/- 2 m globally. This means that the geoid does not coincide exactly with MSL. For example, the geoid is above MSL by about 30 cm in Halifax and below MSL by about 20 cm in Vancouver.
Canadian Geodetic Vertical Datum of 1928 (CGVD28)
CGVD28 is the former vertical datum for Canada. It was adopted by an Order in Council in 1935 and repealed on February 5, 2015. CGVD28 is a tidal datum defined by the mean water level at five tide gauges: Yarmouth and Halifax on the Atlantic Ocean, Pointe-au-Père on the St. Lawrence River, and Vancouver and Prince Rupert on the Pacific Ocean. The definition also includes an elevation at a benchmark in Rouses Point, NY (next to Lake Champlain), which was accepted as fixed by the U.S. and Canada in 1925. The datum is propagated on land using geodetic levelling measurements. The vertical datum is accessible through benchmarks anchored to the ground and stable structures. The heights in terms of CGVD28 are normal-orthometric (Hno).
Vertical control network
The vertical control network consists of some 81,000 first-order benchmarks across Canada. These benchmarks have both CGVD2013 and CGVD28 published heights. The CGVD2013 published heights are, however, considered approximate as they are calculated from historical levelling data and not from the combination of GNSS and a geoid model.
Gravity reference systems
Several networks and satellite missions contribute to Canada’s ability to study gravity and contribute gravity-related data to the CSRS. Read about them below.
Canadian Gravity Standardization Network (CGSN)
Historically the CGSN comprised 6,220 gravity control stations in Canada. The CGSN now has 1,600 control stations distributed throughout Canada, but it is being replaced by a limited number of stations measured accurately by absolute gravimetry. We currently maintain 68 absolute gravity stations distributed across the country. These are co-located with GNSS reference sites, and augmented by 77 other absolute gravity stations that we or the Geological Survey of Canada observed for specific scientific projects. The accuracy of the absolute gravity stations is a few microgals (1 microgal = 10-8 m/s2).
These sites allow us and NRCan partners to conduct scientific studies that include tectonic plate and earthquake deformation, sea-level rise, hydrological mass monitoring (glaciers and water) and glacial isostatic adjustment. The adjustment of the CGSN (original relative network) is based on the International Gravity Standardization Net of 1971 (IGSN71), so the datum definition is considered accurate to several tens of microgals.
Use the CGSN to access data about gravity at any point on the Canadian landmass.
Gravimetry and geodesy
The three pillars of geodesy include the shape of the Earth, its rotation and its gravity field. Gravity measurements help us learn about the Canadian landmass, improve national surveying standards and compute geoid models with respect to the ellipsoid. This enables two things:
- The depiction of the shape of the Earth
- The depiction of the relationship between the GNSS ellipsoidal heights and orthometric heights (heights above MSL)
It also helps us identify potential oil and mineral-bearing geological formations, understand the Earth’s physical processes and define Canada’s continental margins regarding sovereignty in the North.
More reasons to study gravity
For most people, gravity appears as a constant (9.8 m/s2), but in fact, it changes in terms of location and time. That’s because the Earth is not a homogeneous sphere. Gravity changes due to latitude, height and regional mass density. Furthermore, the Earth’s masses are being redistributed continuously and its rotational rate varies ever so slightly. Measuring small changes in gravity allows scientists to better understand the Earth’s physical processes and hydrological cycle.
Variations in the Earth’s gravity can be created by slow processes that take hundreds to billions of years, or processes that take decades or even hours or days. The latter can be observed in earthquakes, for example, and provide us with important information about modern-day climate and environmental changes:
Changes in surface, near-surface or groundwater: This includes small changes in water over large areas that can have a measurable effect on the gravity field. We detect and monitor annual and long-term trends.
Melting glaciers: Many glaciers are melting rapidly and this change in mass distribution has a significant impact on the Earth’s gravity
The moon: The moon’s orbit results in the Earth’s ocean tides and solid Earth tides (the solid Earth deforms as well!). Both of these transient mass redistributions affect gravity.
Earthquakes: Earthquakes redistribute mass locally and change the local gravity field.
Extraction or injection of fluids: The extraction of petroleum products or injection of fluids result in local changes in gravity
Glacial rebound: Regions that were once forced to sink due to the weight of massive overlying glaciers are now, with the glaciers no longer there, slowly returning to their pre-glacial equilibrium. This results in a sizeable vertical uplift across Hudson Bay and Greenland and affects most of Eastern and Northern Canada.
Sea-level change: A rise in sea level adds mass to the oceans and removes it from land, a redistribution of mass that affects the gravity field. In fact, sea-level change does not occur to the same extent everywhere. This is because the surface height of the ocean is determined by local gravity. Significant melting of a glacier will add water to the oceans, globally increasing sea-level on average. But near the glacier, the loss of mass from the glacier will reduce the local gravity field enough that sea-level actually falls. As such, sea-level change and gravity are closely coupled.
Satellite gravimetry
On land, we can measure gravity, gravity difference and gravity variation. In space, four satellite gravity missions are advancing the study of the Earth’s gravity field:
- CHAllenging Minisatellite Payload (CHAMP), 2000–2010
- Gravity Recovery and Climate Experiment (GRACE), 2002–2017
- Gravity Field and Steady-State Ocean Circulation Explorer (GOCE), 2009–2013
- Gravity Recovery and Climate Experiment Follow-on (GRACE-FO), 2018–current
These missions help improve the Canadian geoid model, the basis for Canada’s new height reference system, CGVD2013. Furthermore, satellite gravimetry can measure the temporal variation of the gravity field by monitoring mass redistribution coming from, for example, glacial isostatic adjustment, tectonic plate motions, large earthquakes, glacier melting and hydrological changes (e.g., groundwater variation).
Accurate charting of variations in gravity, either from land, air or space, can be used for numerous purposes:
- Mining exploration: Prospecting for minerals and oil and gas reserves
- Volcanology: Predicting volcanic activity
- Oceanography: Studying and monitoring the state of average MSL to show changes in ocean currents and provide advance flood warnings
- Navigation: Inertial navigation systems of planes, ships and missiles
- Geophysics (studying the Earth’s interior): Providing key information on core and mantle dynamics, which can help explain the Earth’s geology
- Glaciology: Estimating (quite precisely) the thickness of polar ice sheets
- Satellite orbit prediction: Accounting for the impact of gravity on satellite orbits to improve their performance and that of their associated technologies (GNSS)
- Surveying and mapping: Obtaining accurate gravity observations to make a model of MSL (geoid)
- Manufacturing and medicine: Conducting shuttle missions and planned space station research to study cell design and manufacturing processes in a zero-gravity environment